A challenge for a better future: Bringing life back to dead zones
By Arina Favilla, SRC intern
The diversity of life in the ocean, from shallow reefs to deep-sea canyons, is evidence that marine species can successfully adapt to a variety of environments, including low oxygen conditions. For example, in certain places in the world, natural coastal upwelling of nutrients leads to high productivity, which depletes the dissolved oxygen in the water. In these oxygen minimum zones, the benthic fauna have adapted to extremely low levels of dissolved oxygen (0.1 ml of O2/liter) (Diaz et al. 2008). But, what happens when the environment changes drastically in a short period of time? Can the animals adapt fast enough to survive?
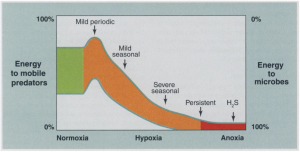
Conceptual graph of how ecosystem energy dynamics change due to changes in oxygen levels. In normal ranges (normoxia), 25-75% of energy is transferred from the benthos to higher-level organisms. As hypoxic conditions emerge, an increased amount of energy is transferred to higher-level organisms. However, the ecosystem cannot support this if hypoxia persists and eventually, microbes process most of the energy as hydrogen sulfide, which further enhances anoxic conditions (Diaz et al. 2008).
In the case of hypoxic dead zones, the answer is usually no. When hypoxic conditions (i.e. low dissolved oxygen) emerge quickly due to unnatural causes, dead zones will likely result. The low oxygen conditions can no longer support life, causing mass mortality and drastic changes in community structure (Diaz et al. 2008). Not only do hypoxic zones cause mortality of benthic fauna, but it also propagates its effects to upper trophic levels, including important commercial species (Vaquer-Sunyer et al. 2008) (Figure 1).
A major culprit contributing to the increase in hypoxic zones worldwide is eutrophication. Eutrophication occurs when river runoff brings excessive amounts of nutrients, such as nitrogen and phosphorous from agriculture fertilizers, into the ocean causing increased primary productivity, such as phytoplankton blooms. When organic matter builds up in the ecosystem faster than it can be decomposed, hypoxic conditions develop. If the physical dynamics of the area promote stratification so that the upper oxygenated water in contact with air does not mix with the bottom water, the region will be especially prone to hypoxia (Diaz 2001).
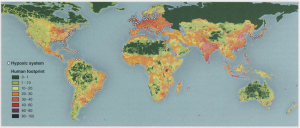
The locations of hypoxic systems match the global human footprint, which is expressed as a normalized percentage, particularly for the Northern Hemisphere where more information is available (Diaz et al. 2008).
Hypoxic zones tend to be around coastal population centers, where the human footprint is greater (Figure 2). The number of dead zones has doubled each decade since the 1960s, now reaching over 400 zones that cover a combined area of 245,000 km2—an area as large as Oregon (Diaz et al. 2008). This is because increased population and living standards have led to agricultural and industrial needs that depend on fertilizers and/or produce other nutrients in the process (Diaz 2001).
While each hypoxic zone is unique in regards to its persistence (e.g. seasonal, periodic, or persistent) and other ambient and physical conditions, a lot can be learned from the successes and failures of one region. Declining oxygen concentrations has been observed in the Black Sea starting in the 1940s and 1950s due to the expansion of agriculture and industry, particularly in the Danube watershed (Diaz et al. 2008, Rabotyagov et al. 2014). The Black Sea hypoxic zone is part of only 8% of dead zones that experience severe, persistent hypoxia and the zone has increased from 3,500 km2 in 1973 to 40,000 km2 in the late 1980s, making it at one point the second largest hypoxic region (Rabotyagov et al. 2014, Diaz et al. 2016). This led to severe problems for the commercial bottom fisheries where only 6 of the 26 highly valued fish species were able to continue to support a fishery (Diaz 2001). It became clear that an intervention was needed—which is exactly what they did. In the early 1990s, the economies bordering the Black Sea agreed to reduce fertilizer use, and by 1995, the hypoxic zone had practically vanished (Rabotyagov et al. 2014). This case exemplifies the effects of excessive nutrients and subsequently reduced nutrient influxes on hypoxic conditions.
Eutrophication is projected to continue to increase, and climate change is expected to further exacerbate the spreading of hypoxic zones through a multitude of pathways. Models predict that climate change may increase stratification and warming, change rainfall patterns, and enhance freshwater river discharge and nutrient flux from agriculture into coastal regions (Diaz et al. 2008, further reading Altieri et al. 2014). Therefore, actions should be taken to limit the expansion of these dead zones. While it is unrealistic to reach nutrient levels of preindustrial times, more regions should attempt to reduce their nutrient inputs in hopes of seeing positive effects similar to that of the Black Sea (Diaz et al. 2008). More importantly, different states and countries must collaborate on the effort—if areas near the coast reduce their nutrient inputs while areas upstream of a river continue to contribute their same nutrient loads, the efforts of the coastal communities would be futile. The importance and sensitivity of this environmental issue is highlighted in the following quote: “There is no other [environmental] variable of such ecological importance to coastal marine ecosystems that has changed so drastically over such a short time as [dissolved oxygen]” (Diaz et al. 2008).
Just as you and I need oxygen to survive, marine organisms require sufficient amounts of dissolved oxygen in the ocean to live. Imagine hiking up to an altitude where it becomes difficult to breathe because the air is “thinner” (i.e. the partial pressure of oxygen is reduced), and feeling the symptoms of altitude sickness, which include those similar to a hangover, but never being able to come back down and recover. When marine populations are starved of oxygen, not only do they suffer, but the entire ecosystem also feels the effects, including us humans who depend on marine resources for food and much more. Therefore, finding a way to bring life back to dead zones and prevent new ones from forming is crucial to preserve our oceans.
Works cited
Altieri, A. H., & Gedan, K. B. 2014. Climate change and dead zones. Global Change Biology 21: 1395-1406.
Diaz, R. J. 2001. Overview of Hypoxia around the World. Journal of Environment Quality 30: 275.
Diaz, R. J., & Rosenberg, R. 2008. Spreading Dead Zones and Consequences for Marine Ecosystems. Science 321: 926-929.
Diaz, R., & Rosenberg, R. 2016. Threats To Coastal Ocean Sustainability: Current Status And Future Trends In Dead Zones. International Seminars on Nuclear War and Planetary Emergencies 48th Session.
Rabotyagov, S. S., Kling, C. L., Gassman, P. W., Rabalais, N. N., & Turner, R. E. 2014. The Economics of Dead Zones: Causes, Impacts, Policy Challenges, and a Model of the Gulf of Mexico Hypoxic Zone. Review of Environmental Economics and Policy 8: 58-79.
Vaquer-Sunyer, R., & Duarte, C. M. 2008. Thresholds of hypoxia for marine biodiversity. Proceedings of the National Academy of Sciences 105: 15452-15457.