By Kevin Reagan, SRC Intern
In recent years, as the effects of global warming and carbon emissions become more and more apparent, the need for renewable energy sources has become more critical than ever. Solar and wind power are popular sources and major investments have been increasing, especially in Europe. But both sources pale in comparison to harnessing the energy contained in moving water, known as hydrokinetic energy, which includes in-stream energy, tidal energy, and wave energy. It is estimated that the global ocean has an energy capacity of 93,100 Terawatt-hours (TWh) per year (one TWh is equivalent to 1 billion kilowatt-hours, or kWh) (Fadaeenejad 346). To put things in perspective, according to the U.S. Energy Information Administration, the average annual electricity consumption for a U.S. residential utility customer in 2014 was 10,932 kWh. In other words, 1 TWh would power roughly 91,000 homes.
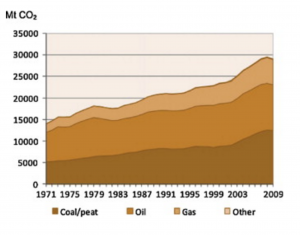
Metric tons of world CO2 emissions from fuel combustion
Although the concept of harnessing hydrokinetic energy has been around for centuries (the first wave energy converter was patented in 1799 and as far back as the 13th century water was used to power mills) it has not been heavily researched until recently (López 414). Hydrostatic technology mainly utilized in dams, has been exploited for a long time in many countries; in the U.S. alone there are over 79,000 dams, and worldwide hydrostatic energy generation is 3,288 TWh (according to the International Energy Agency). Hydrokinetic power on the other hand is cheaper because it is not necessary to build large dams or reservoirs and there is less of an environmental impact. While the power production of a single hydrokinetic device is small-scale, they can be arranged in multi-unit arrays (similar to wind and solar farms) to increase efficiency (Güney 73). While much of the technology is still in the research and development phase, progress is being made, money is being invested, and groups like the Ocean Renewable Energy Coalition in the United States are being established.
There are two main kinds of hydrokinetic energy converters: Current Energy Converters (CECs) and Wave Energy Converters (WECs). CECs are designed to harness energy from a flowing stream (current?) through a rotating turbine, and WECs extract the energy of irregular waves by creating a system of reacting forces in multiple bodies relative to each other (Güney 75). This review will focus mainly on WECs, used to harness tidal and wave energy.
Tidal energy is one of the most easily accessible ocean power sources and can be harnessed as either kinetic energy (of the flowing water) or as potential energy associated with the water level difference between the ebb and flood periods of the tide (Soleimani 77). (Is the way I phrased it correct??) One of the most popular technologies to harness this energy is a tidal barrage situated at the mouth of a basin (bay, estuary, etc.), whose function is similar to a dam. Sluice gates open and allow water to flow into a basin. The gates then close at high tide and the tide outside the gate falls. Once there is enough of a height differential between inside the gates and out, the sluice gates open and water flows out through turbines. This process is known as ebb generation, and produces the most power compared to other current methods (Frid 134).
However, wave energy has by far the most potential for energy production.
It is a much better alternative to fossil fuels than wind and solar energy because its energy density is much higher (2-3 kW/m2 as opposed to 0.4-06 kW/m2 and 0.1-0.2 kW/m2 in wind and solar, respectively), meaning the energy contained in every meter squared of a wave is far greater than that in every meter squared of the wind or solar radiation. WECs can generate power up to 90% of the time (López 414), and it is estimated that there is anywhere from 8,000-80,000 TWh/year in wave energy capacity that can be harnessed depending on the efficiency of the technology (Fadaeenejad 346). In the U.S. alone, there is 2,640 TWh/year of energy in the coastline with 10-50 kW/m transmitted in each wave crest depending on location (Pastor 2). That is enough energy to power every home in America if it could all be harnessed.
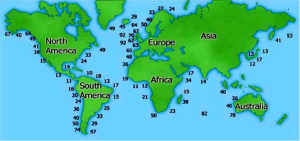
Average amount of energy (in kWh/m) in wave fronts globally
Ocean wave energy comes indirectly from the sun, as solar energy causes wind energy, which results in wave formation, and waves can travel long distance without losing very much energy. Waves are also a dependable power source that can be predicted much better/more easily than wind or solar energy. However, wave power decreases as the wave moves closer to shore due to frictional energy loss to the ocean floor. This means that offshore locations have higher energy potential than nearshore locations, but there is a tradeoff because offshore locations are more difficult to access and maintain and are subject to more extreme weather events, as well as random changes in wave direction (Chandrasekaran 140). The transmission lines would also be more expensive and difficult to maintain.
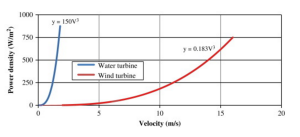
Difference in power density over different media velocities
Since the increase in WEC research, there have been a large number of different kinds of devices developed for various types of locations. As such, these devices have come to be classified in a number of different ways: Location (inshore, nearshore, offshore), method of energy collection, and working principle. There are three main methods of energy collection currently. They are as follows:
- Attenuators– Long structures composed of series of cylindrical sections connected by flexible hinged joints that allow the sections to move relative to each other. They are situated parallel to wave direction.
- Point-Absorbers– Buoy-like structures that collect energy in all direction from the bobbing and pitching of the device.
- Terminators– Long structures situated perpendicular to wave direction that move up and down with the waves and capture or reflect the incoming waves.
There are four main types of working principle for WECs. They are as follows:
- Pressure-differentials– Uses the pressure difference between the crest and trough of a wave to generate power; these are either Archimedes Effect Converters or Oscillating Water Column converters (OWCs)
- Floating Structures– Floating body moved by waves who’s usable back and forth motion can be in multiple directions.
- Overtopping Devices– Force water to pass over the structure and fill an internal reservoir. The water is then release back into the ocean through turbines.
- Impact devices– Flexible structures situated perpendicular to wave motion that move back and forth with the waves.
Some of these different types are summarized in the table below (López 417-419).
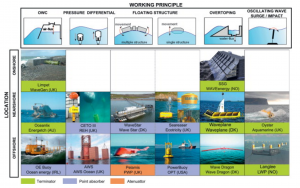
Compilation of several different classifications of WECs
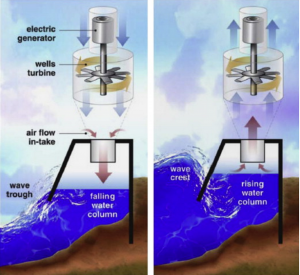
Schematic of a standard OWC converter
Choosing which type of converter is best for a particular location depends on a large number of variables including wave power, water depth, location, and climate; essentially, it is highly variable with some devices being very well suited for some locations and terrible for others. Research has shown that the most energy rich areas are between 40-60˚ latitude in both hemispheres, especially the Southern hemisphere where seasonal variation in weather is lower (López 415). It also depends on the environmental impact of the device. Although the negative environmental impact caused by these devices is minimal, especially when compared to hydrostatic technologies like dams, it is not nonexistent. WECs can alter mixing in the upper layers of the ocean by acting as a wave break, which can remove energy from the wave train and negatively impact ecosystem productivity and will impact reproduction if the currents are altered, as many marine species depend on currents to disperse their larvae. The noise of construction and location of the construction can also have negative environmental impacts by disturbing the species near the area and taking away habitat on the ocean floor, especially tidal barrages (Frid 136-138).
Overall, hydrokinetic technology, especially WECs, have a huge potential for the future of the world. There are several obstacles standing in the way of it becoming a feasible option to power countries, or at least coastal populations, namely lack of funding. However, wave energy also varies with the period and amplitude of the wave and can sometimes be difficult to calculate, which can mean difficulties in choosing the right type of device. As more time and money is devoted to researching new technology and increasing the efficiency of existing technology, we can move closer and closer to powering the world with the abundant, green, and most importantly renewable energy contained in our oceans and rivers. The energy has always been and will always be there; all we need to do is find the best way to exploit it.
Sources:
Chandrasekaran, Srinivasan, Deepak C. Raphel, and Sai Shree. “Deep ocean wave energy systems (DOWES): experimental investigations.” Journal of Naval Architecture and Marine Engineering 11.2 (2014): 139-146.
Fadaeenejad, M., et al. “New approaches in harnessing wave energy: With special attention to small islands.” Renewable and Sustainable Energy Reviews29 (2014): 345-354.
Frid, Chris, et al. “The environmental interactions of tidal and wave energy generation devices.” Environmental Impact Assessment Review 32.1 (2012): 133-139.
Güney, M. S., and K. Kaygusuz. “Hydrokinetic energy conversion systems: A technology status review.” Renewable and Sustainable Energy Reviews 14.9 (2010): 72-82.
López, Iraide, et al. “Review of wave energy technologies and the necessary power-equipment.” Renewable and Sustainable Energy Reviews 27 (2013): 413-434.
Pastor, Jeremiah, and Yucheng Liu. “Hydrokinetic Energy: Overview and it’s Renewable Energy Potential for the Gulf of Mexico.” Green Technologies Conference, 2012 IEEE. IEEE, 2012.
Soleimani, Kaveh, Mohammad Javad Ketabdari, and Farzan Khorasani. “Feasibility study on tidal and wave energy conversion in Iranian seas. “Sustainable Energy Technologies and Assessments 11 (2015): 77-86